DNA

DNA (Deoxyribonucleic acid) provides the genetic information necessary for the development and reproduction of all living organisms and is often described as a genetic blueprint.[1] Every living organism has its own DNA code that is organized in the ladder-like structure of the DNA molecule as a series of complementary paired molecules called bases.
DNA functions as a template: information is transferred from the sequence of base pairs within the DNA, by a molecule called messenger RNA (mRNA), to an enzyme called a ribosome (a biochemical assembly machine). The ribosome translates the code by assembling a protein molecule from amino acids (the building blocks of proteins) according to the base pair sequence brought to it by the mRNA. Proteins are very complex molecules used for signaling, regulation, metabolism and structural components. Cells containing DNA in any one organism have the same DNA, but each different cell type will synthesize its own characteristic combination of proteins, some common to most cells, while others are more specialized and help to define the functions of that particular cell type.
It is the complementary structure of DNA which enables it to function as a template for translating the genetic code and the assembly of the proteins. This complementary structure also enables the double stranded DNA to be separated and replicated as two exact duplicates during cell division (replication). [2]
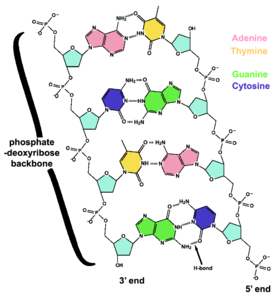
Overview
In most organisms, DNA is formed as a very long and very narrow double-helix formation of two DNA strands coiled around each other in a head-to-toe "antiparallel" orientation. The strands provide structural support for a complementary pair of bases located inbetween the strands (a base pair is like a letter of a genetic word). A sequence of three base pairs forms a codon ( a DNA word) on the DNA strand that encodes the information for one amino acid residue. A series of codons, and associated start/stop codons, (a DNA sentence or gene) forms the genetic code for the selection of particular amino acids and their specific arrangement necessary for the assembly of a particular protein molecule. The protein molecules, as many as 20,000 different types, are used in the cell, or are transported, often via small containers (vesicles), to other areas of the organism.
Each single strand of DNA is a long biopolymer comprised of repeating units called nucleotides. A nucleotide is a base linked to a sugar and phosphate group which form a sugar/phosphate backbone. Attached to each sugar molecule (deoxyribose) is one of the four bases:
The corresponding deoxynucleosides are:
Double stranded DNA is formed by a weak hydrogen bond between the bases holding the nucleotides together. Each base is a structural complement of its opposing base, so both together form the base pair. The complementarity base pairs, A&T, C&G, are identical in size and shape and only one of the four arrangements - TA, AT, GC and CG, will fit between the backbones of double stranded DNA; e.g., adenine always pairs with thymine and guanine always pairs with cytosine. This complementarity, is at work in all DNA functions, and makes it possible for DNA to be copied and repaired relatively easily, while accurately preserving its information content. Thus it forms the basis of semi-conservative DNA replication.
Nuclear DNA is organized and stored as chromosomes within the nucleus. The nucleus is a double membrane separating the DNA from the cytoplasm of the cell enabling certain processing to occur prior to protein synthesis. Each chromosome holds hundreds or thousands of genes. A gene can be described in different ways but in general can be thought of as a whole unit of genetic information.
At conception, the male sperm, (a half stranded DNA with a protein coat), and female ovum, (an unfertilized egg also containing a single strand of DNA), each contribute 23 chromosomes for a total of 46 chromosomes in the fertilized embryo. The total sum of chromosomes is called the kayrotype in eukaryotes (organisms such as plants, yeasts and animals whose cells have a nucleus)
Some organelles in eukaryotic cells (mitochondria and chloroplasts) have their own DNA with a similar organization to bacterial genomes. This DNA encodes for some of the functions in these organelles. Both the mitochondrial DNA (mtDNA) and the chloroplast DNA (cpDNA) originate in the female egg, so the organelle DNA is always inherited from the mother. Some cells, such as blood cells, do not have a nucleus and do not contain DNA. In prokaryote cells (organisms such as common bacteria), DNA is located in a region called the nucleoid. Viruses have a single type of nucleic acid, either DNA or RNA, directly encased in a protein coat called the capsid.
The entire DNA sequence of genes as a whole in any organism is called its genome. The genome provides the necessary genetic instructions to produce the phenotype, the outward physical manifestation of an organism, as well as the necessary processes involved in the replication of nuclear DNA.
Seeking the secret of life
Important genetic discoveries in DNA research spanned many decades. It was taken for granted by early researchers that only a protein molecule was complex enough to contain genetic information. A major discovery included the unexpected realization that the seemingly simple DNA molecule, and not the more complex protein molecule, is responsible for the internally coded, inheritable information defining the genotype. A further significant discovery was that the paired bases exist in equal proportions. These initial discoveries led to the important discovery of the duplex structure of DNA, usually referred to as the "double-helix", that enables the complementary replication of DNA in living organisms. The most important feature of the duplex model is the introduction of the concept of complementarity. Complementarity has come to explain the entire sequence of events in the expression of genetic functions.[3]
Isolating genetic material
DNA was first isolated in 1869 by Friedrich Miescher who discovered a microscopic substance in the pus of discarded surgical bandages. Because it resided in the nuclei of cells, he called it "nuclein".[4] In 1929, Phoebus Levene's identified the base, sugar and phosphate nucleotide unit .[5] Levene suggested that DNA consisted of a string of nucleotide units linked together through the phosphate groups. However Levene thought the chain was short and the bases repeated in a fixed order.
In 1928, Frederick Griffith demonstrated a "transforming factor" that can transmit the ability of Pneumococcus bacteria to cause pneumonia in mice. Although DNA had been identified in cells, it was not considered a strong candidate for the genetic material due to its simple form.
In 1943, Oswald Theodore Avery extended Griffith's work and discovered that traits of the virulent form of Pneumococcus could be transferred to the avirulent form of the same bacteria by mixing killed virulent bacteria with the live avirulent form. Avery identified DNA as the transforming factor.[6] This discovery is considered by some to be a major breakthrough enabling new research methods and a new focus on DNA itself and therefore represents the birth of molecular biology.[7]
DNA's role in heredity was confirmed in 1953, when Alfred Hershey and Martha Chase in the Hershey-Chase experiment, showed that DNA is the genetic material of the T2 phage.[8] This experiment provided the crucial evidence that it was DNA itself, (which was shown to enter the cell), and not a protein,(which remained outside the cell), that was the carrier of information.
Discovering the double helix and code
In 1950, Erwin Chargaff published a paper in which he described something he found extraordinary: the ratios of adenine to thymine were equal, as well as cytosine to guanine. He called this the complementary situation, but later it became known as the Chargaff Ratios. This discovery would play a crucial role in determining the structure of DNA. [9]
As early as 1937 William Astbury produced the first X-ray diffraction patterns that showed that DNA had a regular structure.[10] But it was not until 1953, based on the high quality X-ray diffraction images[11] taken by Rosalind Franklin and the information provided by Erwin Chargaff that the bases were paired, that James D. Watson and Francis Crick constructed a cardboard model that led to [11] what is now accepted as the first accurate model of DNA structure. Their discovery on the morning of February 28, 1953 was reported in the journal Nature.[12]
Experimental evidence for Watson and Crick's model were published in five articles in the same issue of Nature.[13] Of these, Franklin and Raymond Gosling's paper[14] saw the publication of the X-ray diffraction image [15], which was key in Watson and Crick interpretation, as well as another article, co-authored by Maurice Wilkins and his colleagues.[16] Franklin and Gosling's subsequent paper identified the distinctions between the A and B structures of the double helix in DNA.[17] In 1962, Watson, Crick, and Maurice Wilkins jointly received the Nobel Prize in Physiology or Medicine (Franklin didn't share the prize with them as she had died earlier).[18]
In an influential presentation in 1957, Crick laid out the "central dogma" of molecular biology, which foretold the relationship between DNA, RNA, and proteins, and articulated the "adaptor hypothesis".[19] Final confirmation of the replication mechanism that was implied by the double-helical structure followed in 1958 through the Meselson-Stahl experiment.[20] Further work by Crick and coworkers showed that the genetic code was based on non-overlapping triplets of bases, called codons, allowing Har Gobind Khorana, Robert W. Holley and Marshall Warren Nirenberg to decipher the genetic code.[21]
Big science: the Human Genome Project
Initially biologists could only clone and sequence genes one by one, which required large amounts of labor and time in laboratories that were in scattered locations. As technology improved in the 1980's, some scientists such as Craig Venter and Leroy Hood believed their research could focus on sequencing whole genomes and they advocated a era of big science epitomised by the Human Genome Project (HGP). Their goal was to automate the mapping and sequencing to produce resources that would give science a valuable shortcut to addressing the biology of the genome.
Leroy Hood designed DNA sequencing machines to automate the process and thus massively reduce the cost of large scale sequencing. The chemistry of dideoxy sequencing devised by Frederick Sanger in 1977 was still the primary tool but new fluorescent labeling techniques, polymerase chain reaction (PCR) and genomic BAC/YAC libraries, were technological advances that made automation possible. Craig Venter advocated a random "shotgun" approach in the form of sequencing a massive number of randomly picked expressed sequence tags (EST's). Each tag represented a cDNA clone and the whole collection represented a catalog of expressed genes in a genome. This approach transitioned to sequencing random BAC's from the whole genome and was coined shotgun sequencing.
The HGP started out as an academic pursuit that used the traditional and slower target driven approach, a methodological tactic of establishing unique genetic markers, breaking the DNA into smaller bits which were then sequenced and, using the markers as a guide, reassembled. The public consortium was joined by a private effort at Celera Genomics that used the shotgun sequencing approach and a race to finish the project ensued. With the use of computers the vast number of fragments from Celera were assembled using the framework established by the HGP and in June of 2000, the completion of the draft sequence was announced by the leaders of the public and private projects. In January of 2008, the 1000 Genome Project, a mapping of 1000 genomes, was announced.
Assembled according to code
DNA is structured as a tightly-associated repeating pair of molecules (nucleotides) [12][22] entwined in the shape of a double helix. Looking much like a ladder, a typical DNA strand is about 5cm long and only a few atoms in width. The nucleotide repeats contain both the backbone of the molecule, which holds the chain together, and a base, which is a complement of the other DNA strand's base and backbone.
Building blocks
The backbone of the DNA strand has alternating phosphate and sugar residues[23]: the sugar is the pentose (five carbon) sugar 2-deoxyribose. One of the major differences between DNA and RNA is the sugar: 2-deoxyribose is replaced by ribose in RNA.
The sugar molecules are joined together by phosphate groups that form phosphodiester bonds between the third and fifth carbon atoms in the sugar rings. A strand of DNA has a 'direction'. In a double helix, the direction of the nucleotides in one strand is opposite to that in the other strand. Because these bonds are asymmetric, this arrangement of DNA strands is called antiparallel. The asymmetric ends of a strand of DNA bases are referred to as the 5' (five prime) and 3' (three prime) ends.
The double helix is a right-handed spiral. As the DNA strands wind around each other, gaps, called the major and a minor grooves, between the two phosphate backbones reveal the sides of the bases inside (see animation). Two grooves twist around the surface of the double helix: the major groove is 22 Å wide and the minor groove is 12 Å wide.[24] The edges of the bases are more accessible in the major groove, so proteins like transcription factors that can bind to specific sequences in double-stranded DNA usually make contacts to the sides of the bases exposed in the major groove. [25] For many DNA: protein complexes, up to one-half of the contacts involve the DNA phosphate groups, and thus many DNA binding proteins have more positive amino acids (lysine and arginine) than usual.
The double helix is held together by weak hydrogen bonds between the complementary bases attached to the two strands. If many nucleotides are linked together, as in DNA, the polymer is referred to as a polynucleotide.[26]
Base pairing
Each type of base on one strand of DNA forming a bond with a complementary base on the other strand, is called base pairing'. Purines form hydrogen bonds to pyrimidines; for example, A bonds only to T, and C bonds only to G. This arrangement of two nucleotides joined together across the double helix is called a base pair.
The four bases in DNA are adenine (A), cytosine (C), guanine (G) and thymine (T), and these bases are attached to the sugar/phosphate to form the complete nucleotide. Adenine and guanine are fused five- and six-membered heterocyclic compounds called purines, while cytosine and thymine are six-membered rings called pyrimidines.[26] A fifth pyrimidine base, uracil (U), replaces thymine in RNA. Uracil is normally only found in DNA as a breakdown product of cytosine, but bacterial viruses contain uracil in their DNA.[27] In contrast, following synthesis of certain RNA molecules, many uracils are converted to thymines. This occurs mostly on structural and enzymatic RNAs like tRNAs and ribosomal RNA.[28]
In a double helix, the two strands are also held together by hydrophobic effects and by a variation of pi stacking.[29] Hydrogen bonds are weak, and can be broken and rejoined quite easily, so the two strands of DNA in a double helix can be pulled apart like a zipper, either by mechanical force or by high temperatures.[30] Usually the strands are separated by a helicase, a biological unwinding motor. Because the base pairs form a complementarity, the information in the double-stranded sequence of a DNA helix is duplicated on each of the separate complementary opposing strands. The reversible and specific interaction between complementary base pairs is vital in DNA replication. and is critical for all the functions of DNA in living organisms.
The two types of base pairs form different numbers of hydrogen bonds; A&T forms two hydrogen bonds, and G&C forms three, so the G&C base-pair is stronger than the A&T pair. Thus, long DNA helices with a high G&C content have strongly interacting strands, while short helices with high A&T content have weakly interacting strands.[31] Parts of the DNA double helix that need to separate easily tend to have a high A&T content, making the strands easier to pull apart.[32] The strength of this interaction can be measured by finding the temperature required to break the hydrogen bonds (their 'melting temperature'). When all the base pairs in a DNA double helix melt, the strands separate, leaving two single-stranded molecules in solution. Because base-pairing causes changes in the UV-visible spectrum of the bases, DNA melting can be monitored by observing the hypochronicity (or loss thereof) as a function of temperature. These molecules have no single shape, but some conformations are more stable than others.
Flow of information
There are many interactions that happen between DNA and other molecules to coordinate its functions. When cells divide, the genetic information must be duplicated to produce two complementary copies of DNA in a process called DNA replication. When a cell uses the information in a gene, the DNA sequence is copied into a complementary single strand of RNA in a process called transcription. Of the transcribed sequences, some are used to directly make a matching protein sequence by a process called translation (meaning translation from a nucleic acid polymer to an amino acid polymer). The other transcribed RNA sequences may have regulatory, structural or catalytic roles.
Replication
- Further information: Replication of a circular bacterial chromosome
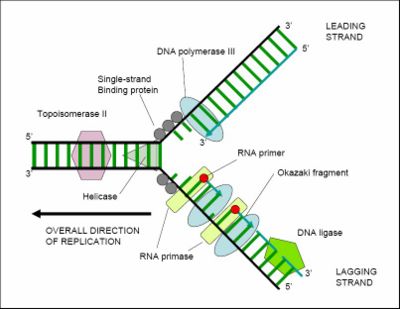
For an organism to grow, its cells must multiply, and this occurs by cell division: one cell splits to create two new 'daughter' cells. When a cell divides it must make an exact copy of its DNA so that the two daughter cells have the same genetic information as the parent cell. DNA replication requires a complex set of proteins, each dedicated to one of several different tasks needed to replicate this large molecule in an orderly and precise fashion. The double-stranded complementary structure of DNA provides a simple mechanism for this replication. The two strands of DNA are separated by a helicase (an unwinding motor), and then each strand's complementary DNA sequence is recreated by an enzyme called DNA polymerase. This makes the complementary strand by finding the correct base (through complementary base pairing), and bonding it to the original strand. DNA polymerases can only extend a DNA strand in a 5' to 3' direction, so other mechanisms are needed to copy the asymetrical antiparallel strand of the double helix.[33] In this way, the base on the old strand dictates which base appears on the new strand, and the cell ends up, after proofreading, with a perfect copy of its DNA.
Further capacity for DNA replication with substantial rearrangement is provided by mechanisms for DNA movement, inversion, and duplication, illustrated by various mobile DNAs such as transposons and proviruses (which has major implications for understanding mechanisms of molecular evolution).
Helicases
Helicases are a type of 'molecular active unwinding motor'[34]used to break hydrogen bonds between bases and unwind the DNA double helix into single strands.[35] Helicases are essential for most processes where enzymes need to access the DNA bases. They use the chemical energy in nucleoside triphosphates (predominantly ATP)
The single-stranded DNA exposed by the activity of the helicase enzyme is quickly bound by single-strand DNA binding proteins. In humans, replication protein A is the best-characterised member of this family, and is essential for most processes where the double helix is separated (including DNA replication, recombination and DNA repair).[36] These proteins seem to stabilize single-stranded DNA and protect it from forming stem loops or being degraded by nucleases.
Topoisomerases
DNA can be 'twisted' in a process called DNA supercoiling. In its "relaxed" state, a DNA strand usually circles the axis of the double helix once every 10.4 base pairs, but if the DNA is twisted, the strands become more tightly or more loosely wound. If the DNA is twisted in the direction of the helix (positive supercoiling), the bases are held more tightly together. If they are twisted in the opposite direction (negative supercoiling) the bases come apart more easily. Topoisomerases have both nuclease and ligase activity, and they can change the amount of supercoiling in DNA. Most DNA has slight negative supercoiling that is introduced by topoisomerases.
These enzymes are needed to relieve the twisting stresses introduced into DNA strands during processes such as transcription and DNA replication.[37]When a section of the double helix is being unwound, the remaining sections can be tangled. Type 1 toppoisomerases work by cutting the DNA helix and allowing one section to rotate; the enzyme then seals the DNA break. Type 11 cuts one DNA helix and then pass a second strand of DNA through this break, before rejoining the helix.[38][39]
Polymerases
The replicative polymerases are required for the faithful replication of the genetic material and they perform this role by attaching the appropriate nucleotide to the nascent strand to match the template strand. [40]Accuracy is very important for this, so many polymerases have a proofreading activity which can recognize the occasional mistakes that can occur during synthesis. These enzymes detect the lack of base pairing between mismatched nucleotides, and if a mismatch is detected, a 3′ to 5′ exonuclease activity is activated and the incorrect base is removed.[41] In most organisms, DNA polymerases function in a large complex called the replisome.
Polymerases synthesise polynucleotides from nucleoside triphosphates. They add nucleotides onto the 3′ hydroxyl group of the previous nucleotide in the DNA strand, so all polymerases work in a 5′ to 3′ direction. In the active site of these enzymes, the nucleoside triphosphate substrate base-pairs to a single-stranded polynucleotide template: this allows polymerases to synthesise the complementary strand of this template accurately.
RNA-dependent DNA polymerases copy the sequence of an RNA strand into DNA. One example is reverse transcriptase, which is a viral enzyme involved in the infection of cells by retroviruses; another example is telomerase, which is required for the replication of telomeres. Telomerase is an unusual polymerase because it contains its own RNA template as part of its structure.[42]
Transcription
Transcription is carried out by a RNA polymerase that copies a DNA sequence into RNA. The enzyme binds to a promoter and separates the DNA strands. It then copies the gene sequence into a mRNA transcript until it reaches a region of DNA called the terminator, where it halts and detaches from the DNA. This complementary copy is then decoded by a ribosome that reads the RNA sequence by base-pairing the mRNA to a specific aminoacyl-tRNA; an amino acid carried by a transfer RNA (tRNA). There is one start codon (AUG, which also encodes for methionine) and three 'stop' or 'nonsense' codons (UAA, UGA and UAG) that signify the end of the coding region.
The genetic code consists of three-letter 'words' called codons formed from a sequence of three nucleotides. As there are four bases in three-letter combinations, there are 64 possible codons, and these encode the twenty standard amino acids. For example, the sequences ACU, CAG and UUU in mRNA are translated to threonine, glutamine and phenylalanine, respectively. Many amino acids, have more than one possible codon (for example, ACU and ACA both code for threonine).
RNA polymerase II, which transcribes most of the genes in the human genome, operates as part of a large protein complex with multiple regulatory and accessory subunits.[43]
Translation
After a gene that encodes a protein is transcribed to a messenger RNA (mRNA), the code is delivered to an RNA/protein complex, an multienzyme, called a ribosome. The code is transferred to the ribosome by tRNA, which then translates the genetic code in a step by step process and assembles a string of amino acids one by one until the a stop codon is reached at which time the completed polypeptide is released into the cytoplasm. Several ribosomes may move along the mRNA simultaneously.
Sense and antisense
When the double strand is separated, two antiparallel strands exist. The DNA sequence that is translated into protein is a "sense" sequence. The sequence on the opposite strand is the "antisense" sequence. Sense and antisense sequences co-exist on the same strand of DNA; in both prokaryotes and eukaryotes, antisense sequences are transcribed[44], and antisense RNAs might be involved in regulating gene expression.[45]. (See Micro RNA, RNA interference, sRNA.)
Nature of genes
DNA contains the genetic information that is the basis for living functions including growth, reproduction and evolution. This information is held in segments of the DNA called genes that may span in size from dozens of DNA base-pairs to many thousands of base-pairs. All humans have essentially the same genes, but have slightly different DNA; on average, the DNA of two individuals differs at about three million bases. These differences are rarely in the protein-coding sequences of genes, but can affect how particular genes are regulated —, exactly where in the body a gene is expressed, how intensely it is expressed, or how expression is regulated by other genes or by environmental factors. These slight differences help to make every human being unique. By comparison, the genome of our closest living relative, the chimpanzee, differs from the human genome by about 30 million bases. [46]
DNA usually occurs as several large, linear chromosomes, each of which may contain hundreds or thousands of genes in eukaryotes. The complete set of chromosomes in a cell makes up its genome; the human genome has about three billion base pairs of DNA arranged into 46 chromosomes [47] and contains 25-30,000 genes.[48] Prokaryotes generally have a single large circular chromosome, but they often possess other miniature chromosomes called plasmids.
The elusive definition
Gene comes from a Greek word meaning "to give birth to". Our understanding of the role genes play in cells, has been continually revised throughout the history of genetics. This has led to several definitions of a gene starting from abstract concepts of inheritable "factors" whose composition was unknown. One way to define a gene is simply as a segment of DNA that is transcribed into RNA - that is - the gene is a unit of transcription. This definition encompasses genes for non-coding RNAs, such as ribosomal RNA (rRNA) and transfer RNA (tRNA), as well as messenger RNA (mRNA) which is used for encoding the sequences of proteins. As defined in this case, a gene then is that whole unit of genetic information which is transcribed and translated into proteins.
A second approach is to define a gene as a region of DNA that encodes a single polypeptide. By this definition, any particular mRNA transcription unit can contain more than one gene, and thus a mRNA can carry regions encoding one or more polypeptides. Such a multi-genic transcription unit is called an operon and are common in prokaryotic cells.Both these definitions are similar to a "bead on a string" analogy with distinct genes as sequentially ordered units along a chromosome.
A more fuzzy definition considers genes as units of biological function. This definition can include cis-acting sequences of DNA that are not transcribed and flank a coding region. They act as specific binding sites for a variety of transcription factor proteins involved with regulating gene expression. Examples of such sequences are enhancers, promoters and operators. In this model the "bead on a string" analogy breaks down since the cis-acting factors from different genes do overlap and this is closer to the reality of gene structure in a chromosome.
Because of these various factors, the exact number of genes in a human organism has not yet been determined.[49]
Expression of genes
How genes are regulated — how they are turned on or off — is an important topic in modern biology, and research continually yields surprising insights into how phenotypic traits and biological adaptations are determined.
In the 1950's, investigations of the bacterium Escherichia coli led to the recognition that the region upstream of a transcribed region provides a place for the enzyme RNA polymerase to attach to DNA and start transcribing RNA in the 5' to 3' direction of the nucleic acid chain. The site at which this occurs came to be called the promoter. Other regulatory proteins (such as repressors) influence transcription by binding to a region near to or overlapping the promoter, called the operator. In the early years of modern genetics, emphasis was given to transcriptional regulation as an important and common means of modulating gene expression, but today we realize that there are a wide range of mechanisms by which the expression of mRNA and proteins can be modulated in cells.
Many DNA sequences in prokaryotes and eukaryotes (and more in plasmids and viruses) have overlapping genes which may both occur in the same direction, on the same strand (parallel) or in opposite directions, on opposite strands (antiparallel).[50][51] In these cases, some DNA sequences encode one protein when read from 5′ to 3′ along one strand, and a different protein when read in the opposite direction (but still from 5′ to 3′) along the other strand. In bacteria, this overlap may be involved in regulating gene transcription,[51] while in viruses, overlapping genes increase the information that can be encoded within the small viral genome.[52] Another way of reducing genome size is seen in some viruses that contain linear or circular single-stranded DNA.[53]
Trans acting proteins
All of the functions of DNA depend on its interactions with proteins. Some of these interactions are non-specific, others are specific in that the protein can only bind to a particular DNA sequence. Some enzymes can also bind to DNA and of these, the polymerases that copy the DNA base sequence in transcription and DNA replication are particularly important.
Other proteins bind to particular DNA sequences. The most intensively studied of these are the transcription factors. Each of these proteins binds to a particular set of DNA sequences and thereby activates or inhibits the transcription of genes with these sequences close to their promoters. Transcription factors do this in two ways. Some can bind the RNA polymerase responsible for transcription, either directly or through other mediator proteins; this locates the polymerase at the promoter and allows it to begin transcription.[54] Other transcription factors can bind enzymes that modify the histones at the promoter, this will change the accessibility of the DNA template to the polymerase.[55]
These DNA targets can occur throughout an organism's genome, so changes in the activity of one type of transcription factor in a given cell can affect the expression of many genes in that cell.[56] Consequently, these proteins are often the targets of the signal transduction processes that mediate responses to environmental changes or cellular differentiation and development. The specificity of transcription factor interactions with DNA arises because the proteins make multiple contacts to the edges of the DNA bases, allowing them to "read" the DNA sequence. Most of these interactions occur in the major groove, where the bases are most accessible.[25]
The chromosome: DNA's home
Nuclear DNA is organized and stored in compacted form into chromosomes inside the nucleus of the cell. At conception, the male sperm and female ovum contribute 23 chromosomes each, for a total of 46 chromosomes in the fertilized embryo. Mitochondria have their own DNA which originates in the female egg as a complete double strand.
DNA is an enormously long, unbranched linear polymer containing many millions of nucleotides the order of which provides the genetic information. For example an average cell has DNA with about 3X109 nucleosides. If each nucleotide were a letter on the page of a large book,they would occupy a million pages. If DNA were stretched out it would measure about 5 cm. but it must be contained in a nucleus measuring 5X10-4 cm in diameter. To achieve the necessary compactness as well as maintain accessibility, DNA is folded in many ways. The folding is assisted by proteins called histones, their sum is known as chromatin. The nucleosome is the first order of folding, appearing as beads on a string under magnification. In this case the DNA wraps around a histone core shaped like a cylinder. The cylinders are then packed together as groups. The groups are then looped. The folding is necessary for maximum compactness and for accessibility of transcription processes.[57]
Histones
![]() |
DNA is held within the chromosomes as a complex of DNA and structural proteins called histones, forming a compact structure called chromatin. In adition to the histones are a variety of proteins called non-histone chromosomal proteins. In prokaryotes many types of proteins are involved.[58] The histones form a disk-shaped complex called a nucleosome which has two complete turns of double-stranded DNA wrapped around it.
These interactions are formed by positively charged amino acid residues in the histones making ionic bonds to the acidic sugar-phosphate backbone of the DNA, and thereby are largely independent of the base sequence.[59] Chemical modifications of these basic amino acid residues include methylation, phosphorylation and acetylation.[60] These changes alter the strength of the interaction between the DNA and the histones, making the DNA more or less accessible to transcription factors and changing the rate of transcription.[61] Other non-specific DNA-binding proteins found in chromatin include the high-mobility group proteins, which bind preferentially to bent or distorted DNA.[62] These proteins are important in bending arrays of nucleosomes and arranging them into more complex chromatin structures.[63]
Enabling transcription
Not only does the chromosome compact DNA, it allows for transcription while in the compacted state.
Genomes
The human genome has about three billion base pairs of DNA arranged into 46 chromosomes[47], and contains 25-30,000 genes [48], the simple nematode C elegans has almost as many genes (more than 19,000)[64]. In eukaryotes, DNA is located mainly in the cell nucleus (there are also small amounts in mitochondria and chloroplasts). In prokaryotes, the DNA is in an irregularly shaped body in the cytoplasm called the nucleoid.[65] The DNA is usually in linear chromosomes in eukaryotes, and circular chromosomes in prokaryotes.
In many species, only a small fraction of the genome encodes protein: only about 1.5% of the human genome consists of protein-coding exons, while over 50% consists of non-coding repetitive sequences.[66] While most of the non-coding elements in human DNA were once considered "junk DNA" with no known function, recent studies suggest a variety of roles for these regions, such as RNA silencing[67] or gene swapping by transposable elements.[68]
Some other vertebrates, including the puffer fish Fugu have more compact genomes, and (for multicellular organisms) there seems to be no consistent relationship between the size of the genome and the complexity of the organism [69]. Some non-coding DNA sequences are now known to have a structural role in chromosomes. In particular, telomeres and centromeres contain few genes, but are important for the function and stability of chromosomes.[70] An abundant form of non-coding DNA in humans are pseudogenes, which are copies of genes that have been disabled by mutation;[71] these are usually just molecular 'fossils', but they can provide the raw genetic material for new genes.[72]
A recent challenge to the long-standing view that the human genome consists of relatively few genes along with a vast amount of "junk DNA" comes from the ENCyclopedia Of DNA Elements (ENCODE) consortium.[73][74] Their survey of the human genome shows that most of the DNA is transcribed into molecules of RNA. This broad pattern of transcription was unexpected, but whether these transcribed (but not translated) elements have any biological function is not yet clear.[74]
The good, the neutral and the ugly
Genetic recombination
A DNA helix does not usually interact with other segments of DNA, and in human cells the different chromosomes even occupy different regions of the nucleus (called "chromosome territories").[75] This physical separation of chromosomes is important for the ability of DNA to function as a stable repository for information, as one of the few times chromosomes interact is when they recombine.
Recombination is when two DNA helices break, swap a section and then rejoin. In eukaryotes, this usually occurs during meiosis, when two chromatids are paired together in the center of the cell. This allows chromosomes to exchange genetic information and produces new combinations of genes.[76] Genetic recombination can also be involved in DNA repair.[77]
The most common form of recombination is homologous recombination, where the two chromosomes involved share very similar sequences. However, recombination can also damage cells, by producing chromosomal translocations and genetic abnormalities. Recombination reactions are catalyzed by recombinases,[78] which have a DNA-dependent ATPase activity. The recombinase makes a 'nick' in one strand of a DNA double helix, allowing the nicked strand to separate from its complementary strand and anneal to one strand of the double helix on the opposite chromatid. A second nick allows the strand in the second chromatid to separate and anneal to the strand in the first helix, forming a cross-strand exchange (also called a Holliday junction). The Holliday junction is a tetrahedral junction structure which can be moved along the pair of chromosomes, swapping one strand for another. The recombination is then halted by cleavage of the junction and re-ligation of the released DNA.[79]
DNA Methylation
DNA methylation is the addition of a methyl group to the fifth carbon of cytosine in effect creating a fifth base. It is a naturally occurring event that can affect many cell functions by inhibiting gene expression, esopecially in the case of foreign DNA. A lack of methylation has been associated with many diseases. On the other hand, methylation of gene p16, a tumor suppressing gene, is suspected to be an epigenetic abnormality occurring early in lung carcinogenesis.[80]
[81] The expression of genes is influenced by the chromatin structure of a chromosome which may hinder or enhance accessibility of the gene. Regions of heterochromatin (with little or no gene expression) correlate with the methylation of cytosine.[82]
These structural changes to the DNA are one type of epigenetic change that can alter chromatin structure, and they are inheritable. Epigenetics refers to features of organisms that are stable over successive rounds of cell division but which do not involve changes in the underlying DNA sequence[83]. Epigenetic changes are important in cellular differentiation, allowing cells to maintain different characteristics despite containing the same genomic material. Some epigenetic features can be inherited from one generation to the next.[84]
Nucleases and ligases
Nucleases cut DNA strands by catalyzing the hydrolysis of the phosphodiester bonds (nucleases that hydrolyse nucleotides from the ends of DNA strands are called exonucleases, while endonucleases cut within strands). The most frequently-used nucleases in molecular biology are the restriction endonucleases, which cut DNA at specific sequences. For instance, the EcoRV enzyme recognizes the 6-base sequence 5′-GAT|ATC-3′ and makes a cut at the vertical line. In nature, these enzymes protect bacteria against phage infection by digesting the phage DNA when it enters the bacterial cell, acting as part of the restriction modification system.[85] In technology, these sequence-specific nucleases are used in molecular cloning and DNA fingerprinting.
DNA ligases can rejoin cut or broken DNA strands, using the energy from either adenosine triphosphate or nicotinamide adenine dinucleotide. Ligases are particularly important in lagging strand DNA replication, as they join together the short segments of DNA produced at the replication fork into a complete copy of the DNA template. They are also used in DNA repair and genetic recombination.[86]
Mutations
DNA can be damaged by many different agents. Mutagens are agents which can produce genetic mutations - these are alterations of one DNA base to another base. Examples of mutagens include oxidizing agents, alkylating agents and high-energy electromagnetic radiation such as ultraviolet light and x-rays.
Lesions of DNA, in which residues are changed to a structure that is not a normal feature of DNA, are different from mutations. However, damaged DNA can give rise to mutations, and indeed, some DNA repair processes are error prone and thus themselves generate mutations. Many mutations have no effect on the function of the genes but some may cause a change in gene expression and function leading to a new phenotype.
Ultraviolet radiation damages DNA mostly by producing thymine dimers.[87] Oxidants such as free radicals or hydrogen peroxide can cause several forms of damage, including base modifications (particularly of guanosine) as well as double-strand breaks.[88] It has been estimated that, in each human cell, about 500 bases suffer oxidative damage every day.[89][90] Of these lesions, the most damaging are double-strand breaks, as they can produce point mutations, insertions and deletions from the DNA sequence, as well as chromosomal translocations.[91]
Many mutagens intercalate into the space between two adjacent base pairs. These are mostly polycyclic, aromatic, and planar molecules, and include ethidium, proflavin, daunomycin, doxorubicin and thalidomide. For an intercalator to fit between base pairs, the bases must separate, distorting the DNA strand by unwinding of the double helix. These structural modifications inhibit transcription and replication processes, causing both toxicity and mutations. As a result, DNA intercalators are often carcinogens. Nevertheless, because they can inhibit DNA transcription and replication, they are also used in chemotherapy to inhibit DNA-replication in rapidly-growing cancer cells.[92]
There are also internal sources for mutagens. Free radicals are produced as a result of cellular respiration and are an unavoidable consequence of living. It is also known that genomes have specific sequences called transposons that have the ability to move around within the genome; they are sometimes referred to as "jumping genes". There are many varieties of transposons, some can break chromosomes or cause deletions, but a common theme is that they cause insertional mutants at their "landing site". [93]
DNA Repair
Environmental factors such as heat and ultraviolet radiation can produce hundreds and even thousands of mistakes in one day. It is crucial that DNA replication accuracy is maintained. Repair is made possible by the complementary structure of DNA. A diverse set of more than 50 repair mechanisms are available to the cell to correct the mistakes. Replication error, after proofreading, is estimated to be one error in 10,000,000,000 replications. [94]
In general the repair process includes the recognition of the damage, uncoiling of the damaged area, snipping out and removing the damaged piece, recreation of the damaged piece using the undamaged complementary as a guide, insertion of the correct nucleotide segment and proofreading the corrections. [95]
An insight into evolution
As well as being susceptible to largely random mutations (that usually affect just a single base), some regions of DNA are specialised to undergo dramatic, rapid, non-random rearrangements, or to undergo more subtle changes at a high frequency so that the expression of a gene is dramatically altered. Such rearrangements include various versions of site-specific recombination. This depends on enzymes that recognise particular sites on DNA and create novel structures such as insertions, deletions and inversions. For instance, DNA regions in the small genome of the bacterial virus P1 can invert, enabling different versions of tail fibers to be expressed in different viruses. Similarly, site-specific recombinase enzymes are responsible for mating type variation in yeast, and for flagellum type (phase) changes in the bacterium Salmonella enterica Typhimurium.
More subtle mutations can occur in micro-satellite repeats (also called homopolymeric tracts of DNA). An example of such a structure are short DNA intervals where the same base is tandemly repeated, as in 5'-gcAAAAAAAAAAAttg-3', or a dinucleotide or even a triplet as in 5'- ATGATGATGATGATGATGATG-3'. DNA polymerase III is prone to make 'stuttering errors' at such repeats. As a consequence, changes in repeat number occur quite often during cell replication, and when they appear at a position where the spacing of nucleotide residues is critical for gene function, they can cause changes to the phenotype.
The stomach ulcer bacterium Helicobacter pylori is a good example of how homopolymeric tracts can enable quasi-directed evolution of particular genes. H. pylori has 46 genes that contain homopolymeric runs of nucleotides or dinucleotide repeats that are prone to frequent length changes as a consequence of stuttering errors during replication. These changes can lead to frequent reversible inactivation of these genes, or to changed gene transcription if the repeat is located in a regulatory sequence. This generates highly diverse populations of H. pylori in an individual human host, and this diversity helps the bacterium evade the immune system.[96]
Triplet repeats behave similarly, but are particularly suited to evolution of proteins with differing characteristics. In the clock-like period gene of the fruit fly, triplet repeats 'fine tune' an insect's biological clock in response to changes in environmental temperatures. Triplet repeats are widely distributed in genomes, and their high frequency of mutation is responsible for several genetically determined disorders in humans.[97]
Impact on society
Forensics
Forensic scientists can use DNA in blood, semen, skin, saliva or hair to match samples collected at a crime scene to samples taken from possible suspects. This process is called genetic fingerprinting or more formally, DNA profiling. In DNA profiling, the lengths of variable sections of repetitive DNA (such as short tandem repeats and minisatellites) are compared between people. This is usually very reliable for identifying the source of a sample,[98] but identification can be complicated if the samples that are collected include DNA from several people.[99]
DNA profiling was developed in 1984 by British geneticist Sir Alec Jeffreys,[100] and first used in forensic science to convict Colin Pitchfork (and to clear the prime suspect) in the 1988 Enderby murders case.[101] People convicted of certain types of crimes may be required to provide a sample of DNA for a database. This has helped investigators solve old cases where only a DNA sample was obtained from the scene. DNA profiling can also be used to identify victims of mass casualty incidents.[102]
Bioinformatics
One branch of bioinformatics involves the analysis of DNA sequence data; DNA from hundreds of different organisms has now been partially sequenced, and this information is stored in massive databases. The development of techniques to store and search DNA sequences has led to many applications in computer science.[103]
String-searching or 'matching' algorithms, which identify a given sequence of letters inside a larger sequence of letters, are used to search for specific sequences of nucleotides. The related problem of sequence alignment aims to identify homologous sequences; these are sequences that are very similar but not identical. When two different genes in an organism have very similar sequences, this is evidence that, at some stage in evolution, a single gene was duplicated, and the sequences subsequently diverged (under different selection pressures) by incorporating different mutations. Identifying such holologies can give valuable clues about the likely function of novel genes. Similarly, identifying homologies between genes in different organisms can be used to reconstruct the evolutionary relationships between organisms.[104] Data sets representing entire genomes' worth of DNA sequences, such as those produced by the Human Genome Project, are difficult to use without annotations, which label the locations of genes and regulatory elements on each chromosome. Regions of DNA sequence that have patterns that are characteristic of protein- or RNA-coding genes can be identified by gene finding algorithms, allowing researchers to predict the presence of particular gene products in an organism.[105]
Molecular cloning
The small chromosomes of bacteria have proved extraordinarily useful for analysing genetic mechanisms and genome structure in many organisms, including humans. This utility arose because of several important discoveries made by microbiologists in the 1950-70's that provided new laboratory tools for directly manipulating genes. The principal laboratory tools and techniques discovered in this period were:
- Compact circular plasmid molecules such as ColE1 that were relatively easy to extract and purify from bacterial cells, and when inserted back inside living bacterial cells could serve as genetically stable carriers (vectors) of novel DNA fragments. These vectors were propagated in bacterial cell lines termed "clones". Since each plasmid vector in these clones carried a single DNA molecular fragment, these could be considered to be 'molecular clones'.
- Convenient methods for extracting plasmid DNA from cells, physical analysis of this DNA, and reinsertion of circular plasmid DNA back inside living cells. The main method for DNA re-insertion is termed DNA transformation - that is, direct uptake of naked DNA molecules by cells. One technique that assisted DNA transformation was the use of selectable genetic markers, and plasmid-borne bacterial antibiotic resistance provided such markers in the form of traits like ampicillin resistance and tetracycline resistance.
- Restriction endonucleases such as the enzymes EcoRI or HindIII, were found to be useful for specifically digesting DNA at particular sites and creating novel combinations of DNA fragments in the laboratory.
- Restriction enzymes enabled re-annealing different DNA fragments, such as circular plasmid DNA which had been linearised at a single EcoRI restriction endonuclease target site together with another fragment, say an EcoRI generated fragment of a human chromosome. By sealing together two such fragments with the enzyme DNA ligase, novel hybrid-plasmid molecules could be created in which "foreign" DNA inserts were carried in a chimeric or hybrid plasmid, often called a recombinant DNA molecule.
After the mid-1970's, re-insertion of recombinant DNA plasmids into living bacterial cells, such as those of Escherichia coli opened up many new approaches in biotechnology. These made it possible to manufacture, for example, human hormones such as insulin in microbial cell-based protein factories. When combined with other genetic techniques, the field of "recombinant DNA technology" opened up the possibility of decoding the gene sequence of whole genomes. This latter area is now usually referred to as genomics, and includes the Human Genome Project.
Bringing it together
On January 28, 2008, an international research consortium announced the 1000 Genomes Project involving sequencing the genomes of at least a thousand people from around the world to create the most detailed and medically useful picture to date of human genetic variation. Drawing on the expertise of multidisciplinary research teams, the 1000 Genomes Project will develop a new map of the human genome that will provide a view of biomedically relevant DNA variations at a resolution unmatched by current resources.
“The 1000 Genomes Project will examine the human genome at a level of detail that no one has done before,” said Richard Durbin, Ph.D., of the Wellcome Trust Sanger Institute, who is co-chair of the consortium. “Such a project would have been unthinkable only two years ago. Today, thanks to amazing strides in sequencing technology, bioinformatics and population genomics, it is now within our grasp. So we are moving forward to build a tool that will greatly expand and further accelerate efforts to find more of the genetic factors involved in human health and disease.”
As with other major human genome reference projects, data from the 1000 Genomes Project will be available to the worldwide scientific community through freely accessible public databases.
One example of the impact on understanding the biological basis for schizophrenia, was reported in Scientific American.[106]
The researchers determined which affected genes were likely to cause damage—and where in the body that damage that might occur. The flawed genes in the schizophrenia patients were overwhelmingly linked to changes in pathways responsible for communication between and within nerve cells. In fact, one gene, ERBB4, is known to code for a receptor that interacts with neuregulin 1, a protein that's been associated with the illness for a decade. 'The silver lining is that these new findings show us … that we were already on the right track,' Sebat says."
Some researchers believe that future advances in genetics will result in patient specific treatments, however, they also express concerns about the cost of such drugs which will be needed only by a few. Recent advances also include the capability to take a skin cell of a patient with a disease, transform it into a stem cell, and then program it to grow into the particular kind of cell which is affected by the disease which then can be studied.
Looking to the Future
What may be of great significance and importance to biology and science in general is the realization made clear by the Genome Project that knowing merely the sequence of events is not enough to understand how life works. Also made clear is the realization that a tremendous amount of information is contained not only in the objects of study but also in the relationships that they maintain. As a result, a new discipline has emerged in the form of "Systems Biology" [107] An integrative system is a perspective defined by biologist Ludwig von Bertalanffy as elements in standing relationship taken as a whole.[108] Systems Biology complements the traditional "mechanistic" methodology of investigating isolated entities with a further perspective focusing on the organization of those entities. [109]
References
- ↑ All living organisms use DNA as their genetic material with the exception of some RNA viruses. Whether viruses are alive is also a debatable question among biologists since they do not qualify based on some the the prerequisites for life, such as having a metabolism].
- ↑ Kornberg, Arthur. DNA Replication. W.H.Freeman and Co. (1980) "Complementarity has come to explain transcription and translation and thus the entire sequence of events in the expression of genetic functions. It is also the basis for exchange of DNA segments between chromosomes in several forms of recombination."
- ↑ Kornberg, Arthur. DNA Replication. W.H.Freeman and Co. (1980) p13
- ↑ Dahm R (2005). "Friedrich Miescher and the discovery of DNA". Dev Biol 278: 274-88. PMID 15680349.
- ↑ Levene P (1919). "The structure of yeast nucleic acid". J Biol Chem 40: 415-24.
- ↑ Avery O, MacLeod C, McCarty M (1979). "Studies on the chemical nature of the substance inducing transformation of pneumococcal types. Inductions of transformation by a desoxyribonucleic acid fraction isolated from pneumococcus type III". J Exp Med 149 (2): 297-326. PMID 33226.
- ↑ Asimov, Isaac. (1962) The Genetic Code. pg viii New American Library. Written while he was Associate Professor of Biochemistry at Boston University School of Medicine. "It launched in a new direction the investigation into the nature of life and brought about a new research methods. The branch of science now called "molecular biology" came into its own."
- ↑ Hershey A, Chase M (1952). "Independent functions of viral protein and nucleic acid in growth of bacteriophage". J Gen Physiol 36 (1): 39-56. PMID 12981234.
- ↑ Interview of Chargaff at DNA interactive web site.
- ↑ Astbury W (1947). "Nucleic acid". Symp Soc Exp Biol 1.
- ↑ 11.0 11.1 Watson JD, Crick FHC (1953) A structure for deoxyribose nucleic acid. 'Nature 171:737–8 Accessed 13 Feb 2007.
- ↑ 12.0 12.1 Watson J, Crick F (1953). "Molecular structure of nucleic acids; a structure for deoxyribose nucleic acid". Nature 171: 737-8. PMID 13054692.
- ↑ Nature Archives Double Helix of DNA: 50 Years
- ↑ Franklin R, Gosling RG (1953)Molecular configuration in sodium thymonucleate. Nature N 171, 740–1
- ↑ Original X--ray diffraction image
- ↑ Wilkins MHF, Stokes AR, Wilson HR (1953) Molecular structure of deoxypentose nucleic acids.Nature [http://www.nature.com/nature/dna50/wilkins.pdf 171:738–40
- ↑ Franklin R, Gosling RG (1953) Evidence for 2-chain helix in crystalline structure of Sodium Deoxyribonucleate. Nature 172:156–7
- ↑ The Nobel Prize in Physiology or Medicine 1962 Nobelprize .org Accessed 22 Dec 06
- ↑ Crick FHC (1955) On degenerate templates and the adaptor hypothesis(PDF) genome.wellcome.ac.uk (Accessed 22 Dec 2006
- ↑ Meselson M, Stahl F (1958). "The replication of DNA in Escherichia coli". Proc Natl Acad Sci USA 44: 671-82. PMID 16590258.
- ↑ The Nobel Prize in Physiology or Medicine 1968 Nobelprize.org Accessed 22 Dec 06
- ↑ Berg J et al. (2002) Biochemistry. WH Freeman and Co. ISBN 0-7167-4955-6
- ↑ Ghosh A, Bansal M (2003). "A glossary of DNA structures from A to Z". Acta Crystallogr D Biol Crystallogr 59: 620-6. PMID 12657780.
- ↑ Wing R et al. (1980). "Crystal structure analysis of a complete turn of B-DNA". Nature 287: 755–8. PMID 7432492.
- ↑ 25.0 25.1 Pabo C, Sauer R. "Protein-DNA recognition". Ann Rev Biochem 53: 293-321. PMID 6236744.
- ↑ 26.0 26.1 Abbreviations and Symbols for Nucleic Acids, Polynucleotides and their Constituents IUPAC-IUB Commission on Biochemical Nomenclature (CBN) Accessed 03 Jan 2006
- ↑ Takahashi I, Marmur J (1963). "Replacement of thymidylic acid by deoxyuridylic acid in the deoxyribonucleic acid of a transducing phage for Bacillus subtilis". Nature 197: 794-5. PMID 13980287.
- ↑ Agris P (2004). "Decoding the genome: a modified view". Nucleic Acids Res 32: 223 – 38. PMID 14715921.
- ↑ Ponnuswamy P, Gromiha M (1994). "On the conformational stability of oligonucleotide duplexes and tRNA molecules". J Theor Biol 169: 419-32. PMID 7526075.
- ↑ Clausen-Schaumann H et al. (2000). "Mechanical stability of single DNA molecules". Biophys J 78: 1997-2007. PMID 10733978.
- ↑ Chalikian T et al. (1999). "A more unified picture for the thermodynamics of nucleic acid duplex melting: a characterization by calorimetric and volumetric techniques". Proc Natl Acad Sci USA 96: 7853-8. PMID 10393911.
- ↑ deHaseth P, Helmann J (1995). "Open complex formation by Escherichia coli RNA polymerase: the mechanism of polymerase-induced strand separation of double helical DNA". Mol Microbiol 16: 817-24. PMID 7476180.
- ↑ Albà M (2001). "Replicative DNA polymerases". Genome Biol 2: REVIEWS3002. PMID 11178285.
- ↑ http://www.news.cornell.edu/stories/July07/helicases.kr.html
- ↑ Tuteja N, Tuteja R (2004). "Unraveling DNA helicases. Motif, structure, mechanism and function". Eur J Biochem 271: 1849–63. PMID 15128295.
- ↑ Iftode C et al. (1999). "Replication protein A (RPA): the eukaryotic SSB". Crit Rev Biochem Mol Biol 34: 141-80. PMID 10473346.
- ↑ Wang J (2002). "Cellular roles of DNA topoisomerases: a molecular perspective". Nat Rev Mol Cell Biol 3: 430–40. PMID 12042765.
- ↑ Schoeffler A, Berger J (2005). "Recent advances in understanding structure-function relationships in the type II topoisomerase mechanism". Biochem Soc Trans 33: 1465–70. PMID 16246147.
- ↑ Champoux J. "DNA topoisomerases: structure, function, and mechanism". Ann Rev Biochem 70: 369–413. PMID 11395412.
- ↑ http://www.pubmedcentral.nih.gov/articlerender.fcgi?artid=150442
- ↑ Hubscher U et al.. "Eukaryotic DNA polymerases". Annu Rev Biochem 71: 133–63. PMID 12045093.
- ↑ Nugent C, Lundblad V (1998). "The telomerase reverse transcriptase: components and regulation". Genes Dev 12: 1073–85. PMID 9553037.
- ↑ Martinez E (2002). "Multi-protein complexes in eukaryotic gene transcription". Plant Mol Biol 50: 925–47. PMID 12516863.
- ↑ Hüttenhofer A et al. (2005). "Non-coding RNAs: hope or hype?". Trends Genet 21: 289-97. PMID 15851066.
- ↑ Munroe S (2004). "Diversity of antisense regulation in eukaryotes: multiple mechanisms, emerging patterns". J Cell Biochem 93: 664-71. PMID 15389973.
- ↑ Pollard KS et al. (2006) Forces shaping the fastest evolving regions in the human genome. PLoS Genet 2:e168 PMID 17040131
- ↑ 47.0 47.1 Venter J et al. (2001). "The sequence of the human genome". Science 291: 1304–51. PMID 11181995.
- ↑ 48.0 48.1 Human Genome Project Information [1]
- ↑ Insert footnote text here
- ↑ Makalowska I et al. (2005). "Overlapping genes in vertebrate genomes". Comput Biol Chem 29: 1–12. PMID 15680581.
- ↑ 51.0 51.1 Johnson Z, Chisholm S (2004). "Properties of overlapping genes are conserved across microbial genomes". Genome Res 14: 2268–72. PMID 15520290.
- ↑ Lamb R, Horvath C (1991). "Diversity of coding strategies in influenza viruses". Trends Genet 7: 261–6. PMID 1771674.
- ↑ Davies J, Stanley J (1989). "Geminivirus genes and vectors". Trends Genet 5: 77–81. PMID 2660364.
- ↑ Myers L, Kornberg R. "Mediator of transcriptional regulation". Ann Rev Biochem 69: 729-49. PMID 10966474.
- ↑ Spiegelman B, Heinrich R (2004). "Biological control through regulated transcriptional coactivators". Cell 119: 157-67. PMID 15479634.
- ↑ Li Z et al. (2003). "A global transcriptional regulatory role for c-Myc in Burkitt's lymphoma cells". Proc Natl Acad Sci USA 100: 8164-9. PMID 12808131.
- ↑ Insert footnote text here
- ↑ Sandman K et al. (1998). "Diversity of prokaryotic chromosomal proteins and the origin of the nucleosome". Cell Mol Life Sci 54: 1350-64. PMID 9893710.
- ↑ Luger K et al. (1997). "Crystal structure of the nucleosome core particle at 2.8 A resolution". Nature 389: 251-60. PMID 9305837.
- ↑ Jenuwein T, Allis C (2001). "Translating the histone code". Science 293: 1074-80. PMID 11498575.
- ↑ Ito T. "Nucleosome assembly and remodelling". Curr Top Microbiol Immunol 274: 1-22. PMID 12596902.
- ↑ Thomas J (2001). "HMG1 and 2: architectural DNA-binding proteins". Biochem Soc Trans 29: 395-401. PMID 11497996.
- ↑ Grosschedl R et al. (1994). "HMG domain proteins: architectural elements in the assembly of nucleoprotein structures". Trends Genet 10: 94-100. PMID 8178371.
- ↑ The C. elegans Sequencing Consortium (1998)Genome Sequence of the Nematode C. elegans: A Platform for Investigating Biology. Science 282: 2012-8 [2]
- ↑ Thanbichler M et al. (2005). "The bacterial nucleoid: a highly organized and dynamic structure". J Cell Biochem 96: 506–21. PMID 15988757.
- ↑ Wolfsberg T et al. (2001). "Guide to the draft human genome". Nature 409: 824-6. PMID 11236998.
- ↑ Volpe, TA "et al." (2002). "Regulation of heterochromatic silencing and histone H3 lysine-9 methylation by RNAi". Science 297: 1833-1837.
- ↑ Biemont C, Vieira C (2006). "Genetics: Junk DNA as an evolutionary force". Nature 443: 521-4.
- ↑ See Sydney Brennar's Nobel lecture (2002) [3]
- ↑ Pidoux A, Allshire R (2005). "The role of heterochromatin in centromere function". Philos Trans R Soc Lond B 360: 569-79. PMID 15905142.
- ↑ Harrison P et al. (2002). "Molecular fossils in the human genome: identification and analysis of the pseudogenes in chromosomes 21 and 22". Genome Res 12: 272-80. PMID 11827946.
- ↑ Harrison P, Gerstein M (2002). "Studying genomes through the aeons: protein families, pseudogenes and proteome evolution". J Mol Biol 318: 1155-74. PMID 12083509.
- ↑ Weinstock GM (2007). "ENCODE: More genomic empowerment". Genome Research 17: 667-668. PMID 17567987.
- ↑ 74.0 74.1 ENCODE Project Consortium (2007). "Identification and analysis of functional elements in 1% of the human genome by the ENCODE pilot project". Nature 447: 799-816. PMID 17571346.
- ↑ Cremer T, Cremer C (2001). "Chromosome territories, nuclear architecture and gene regulation in mammalian cells". Nat Rev Genet 2: 292-301. PMID 11283701.
- ↑ Pál C et al. (2006). "An integrated view of protein evolution". Nat Rev Genet 7: 337-48. PMID 16619049.
- ↑ O'Driscoll M, Jeggo P (2006). "The role of double-strand break repair - insights from human genetics". Nat Rev Genet 7: 45-54. PMID 16369571.
- ↑ Sung P et al. (2003). "Rad51 recombinase and recombination mediators". J Biol Chem 278: 42729-32. PMID 12912992.
- ↑ Dickman M et al. (2002). "The RuvABC resolvasome". Eur J Biochem 269: 5492-501. PMID 12423347.
- ↑ http://cancerres.aacrjournals.org/cgi/content/abstract/62/2/351
- ↑ Blood, Vol. 93 No. 12 (June 15), 1999: pp. 4059-4070 online: http://bloodjournal.hematologylibrary.org/cgi/content/full/93/12/4059#Top
- ↑ For example, cytosine methylation, to produce 5-methylcytosine, is important for X-chromosome inactivation. Klose R, Bird A (2006). "Genomic DNA methylation: the mark and its mediators". Trends Biochem Sci 31: 89–97. PMID 16403636.
- ↑ Bird A (2007). "Perceptions of epigenetics". Nature 447: 396-8. PMID 17522671
- ↑ Chandler VL (2007). "Paramutation: from maize to mice". Cell 128: 641-5.
- ↑ Bickle T, Krüger D (1993). "Biology of DNA restriction". Microbiol Rev 57: 434–50. PMID 8336674.
- ↑ Doherty A, Suh S (2000). "Structural and mechanistic conservation in DNA ligases.". Nucleic Acids Res 28: 4051–8. PMID 11058099.
- ↑ Douki T et al. (2003). "Bipyrimidine photoproducts rather than oxidative lesions are the main type of DNA damage involved in the genotoxic effect of solar ultraviolet radiation". Biochemistry 42: 9221-6. PMID 12885257.
- ↑ Cadet J et al. (1999). "Hydroxyl radicals and DNA base damage". Mutat Res 424 (1-2): 9-21. PMID 10064846.
- ↑ Shigenaga M et al. (1989). "Urinary 8-hydroxy-2'-deoxyguanosine as a biological marker of in vivo oxidative DNA damage". Proc Natl Acad Sci USA 86: 9697-701. PMID 2602371.
- ↑ Cathcart R et al. (1984). "Thymine glycol and thymidine glycol in human and rat urine: a possible assay for oxidative DNA damage". Proc Natl Acad Sci USA 81: 5633-7. PMID 6592579.
- ↑ Valerie K, Povirk L (2003). "Regulation and mechanisms of mammalian double-strand break repair". Oncogene 22: 5792-812. PMID 12947387.
- ↑ Braña M et al. (2001). "Intercalators as anticancer drugs". Curr Pharm Des 7: 1745-80. PMID 11562309.
- ↑ I think we need a more general review of all transposons here not just retrotransposons
- ↑ Hanawalt, P.C., and Setlow, R.B. (eds) Molecular Mechanisms for Repair of DNA. Plenum Press New York.
- ↑ 6 de Boer J, Hoeijmakers JH. Nucleotide excision repair and human syndromes. Carcinogenesis 2000; 21: 453-60. online: http://websites.afar.org/site/PageServer?pagename=IA_b_dna_6_r_proc
- ↑ Suerbaum S, Josenhans C (2007) Helicobacter pylori evolution and phenotypic diversification in a changing host. Nat Rev Microbiol 5:441-52 PMID 17505524
- ↑ Christopher Wills discusses the evolutionary significance of these concepts in The Runaway Brain: The Evolution of Human Uniqueness ISBN 0-00-654672-2 (1995)
- ↑ Collins A, Morton N (1994). "Likelihood ratios for DNA identification". Proc Natl Acad Sci USA 91: 6007-11. PMID 8016106.
- ↑ Weir B et al. (1997). "Interpreting DNA mixtures". J Forensic Sci 42: 213-22. PMID 9068179.
- ↑ Jeffreys A et al. (1985). "Individual-specific 'fingerprints' of human DNA.". Nature 316: 76-9. PMID 2989708.
- ↑ Colin Pitchfork - first murder conviction on DNA evidence also clears the prime suspect Forensic Science Service Accessed 23 Dec 2006
- ↑ DNA Identification in Mass Fatality Incidents. National Institute of Justice (September 2006).
- ↑ Baldi, Pierre. Brunak, Soren. Bioinformatics: The Machine Learning Approach MIT Press (2001) ISBN 978-0-262-02506-5
- ↑ Sjölander K (2004). "Phylogenomic inference of protein molecular function: advances and challenges". Bioinformatics 20: 170-9. PMID 14734307.
- ↑ Mount DM (2004). Bioinformatics: Sequence and Genome Analysis, 2. Cold Spring Harbor Laboratory Press. ISBN 0879697121.
- ↑ Nikhil Swaminathan, A New, Genetic Model for Schizophrenia Scientific American 2008, March 28.
- ↑ Drack, Manfred., Apfalter, Wilfried., Pouvreau, David. (2007) On the Making of a System Theory of Life: Paul A. Weiss and Ludwig von Bertalanffy's Conceptual Connection. The Quarterly Review of Biology 82(4), The University of Chicago, Chicago.
- ↑ Bertalanffy, Ludwig von,. (1968) General System Theory. George Braziller, N.Y., N.Y..
- ↑ Pouvreau, David. Drack, Manfred. (2007) On the History of Ludwig von Bertalanffy's "General Systemology", and on its relationship to cybernetics. International Journal of General Systems Vol. 36, No. 3, June 2007, 282-337. Taylor & Francis ISSN 0408-1079 (print) ISSN 1563-5104 (online)